INTRODUCTION
Fatty Acid Binding Proteins (FABPs) are vital for fatty acid transport and metabolism, influencing fat storage and distribution (Zhang et al., 2020). They not only metabolize long-chain fatty acids (LCFAs) but also act as signaling molecules, impacting signaling pathways and gene expression (Yang et al., 2011). In vertebrates, there are 12 FABP types, with the chicken genome containing nine (Chmurzyńska, 2006; Zhang et al., 2020). FABP genes are expressed in various tissues, including muscle, adipose tissue, liver, intestine, myelin, and epidermis (Chmurzyńska, 2006; Cahyadi et al., 2013). Fatty Acid-Binding Protein 4 (FABP4) is an adipocyte-specific protein, highly expressed in subcutaneous adipose tissues (Gardan et al., 2007; Cahyadi et al., 2013). It aids in fatty acid transport and oxidation and is associated with adipose tissue inflammation, synergizing with leptin (Gan et al., 2015). FABP4 enhances LCFA solubility and facilitates their transport between cellular membranes and organelles (Coburn et al., 2000; Gan et al., 2015). Its activity can be influenced by factors like LCFAs, oxidized low-density lipoprotein, peroxisome proliferator-activated receptor γ (PPARγ), and insulin (Lapsys et al., 2000; Gan et al., 2015). Previous studies report the role of FABP4 in chicken physiology, impacting body weight (Cahyadi et al., 2013), fat mass expandability (Berger and Géloën, 2023), and leptin regulation (Gan et al., 2015). It serves as a biomarker of lipogenesis and has links to inflammation and immunity. In macrophages, FABP4 regulates inflammatory responses through the IKK—nuclear factor-κβ (NF-κβ) pathway (Amiri et al., 2018). Suppressing FABP4 reduces inflammation, oxidative stress, and apoptosis by inhibiting NF-κβ and activating PPARγ (Mao et al., 2021) Additionally, in acute kidney injury, increased FABP4 exacerbates inflammation and apoptosis via TLR4/c-Jun signaling activation (Wang et al., 2022).
The immune system responds to the invasion of bacteria, viruses, or external substances by activating innate immune receptors, such as Toll-like receptors (TLRs) and Nod-like receptors (NLRs), which recognize characteristic molecular patterns of the microbes or derivatives. This leads to the production of inflammatory cytokines and the regulation of the transcription of innate immune receptor genes, thereby initiating innate immune responses. Toll-like receptors are transmembrane proteins that are activated upon recognizing pathogen-associated molecular patterns (PAMPs), including bacterial DNA, viral double/single-stranded RNA, lipopolysaccharides, peptidoglycans, and flagellin (Nawab et al., 2019).
RNA viruses like the avian influenza virus (AIV) trigger a host immune response through the TLR3 receptor within endosomes. This activation leads to NF-κB transcription factor activation, which, under normal conditions, is inhibited by IκB in the cytoplasm. Once activated, NF-κB regulates gene transcription in the nucleus. TLR3’s activation initiates a cascade, resulting in cytokine and chemokine production, attracting immune cells to the infection site, and sparking inflammation. This response is vital for controlling viral infections and preventing their spread. Elevated TLR3 and cytokine levels during viral infection signify the initiation of a protective immune response. Studying TLR3 regulation in chickens can inform strategies to combat viral infections in poultry (Cheng et al., 2014; Zhang et al., 2015).
Recent studies have recognized FABP4 as a biomarker for chicken lipogenesis and chronic inflammation. However, Research on the signaling pathways affecting chFABP4 expression in chickens is limited. Therefore, our investigation focused on chFABP4 expression when exposed to TLR3 signals. We also assessed the transcriptional regulation of chFABP4 using inhibitors for nuclear factor κB (NF-κB) and mitogen-activated protein kinases (MAPKs), namely, p38, c-Jun-N-terminal kinase (JNK), and mitogen-activated protein kinase kinase (MEK), in chicken DF-1 cells.
MATERIALS AND METHODS
The chicken cell line DF-1 (ATCC, CRL-12203) was purchased from the American Tissue Culture Collection (ATCC, Manassas, VA, USA). DF-1 cells were grown in Dulbecco’s modified Eagle’s medium (DMEM), purchased from Biowest (Riverside, MO, USA), supplemented with 10% fetal bovine serum (FBS), 2 M L-glutamine and 100 U/mL each of penicillin and streptomycin (Thermo Fisher Scientific, Waltham, MA, USA) at 37°C in a humidified atmosphere of 5% CO2 and 95% air.
Bay 11-7085 (Bay) was procured from Sigma-Aldrich (St. Louis, MO, USA). Polyinosinic:polycytidylic acid (PIC), SB203580 (p38 inhibitor), SP600125 (JNK inhibitor) were acquired from Invivogen (San Diego, CA, USA), and PD98059 (MEK inhibitor) was obtained from Medchem Express (Monmouth Junction, NJ, USA). These inhibitors were stored in accordance with the manufacturer’s instructions. The inhibitors were applied to DF-1 cells at the following doses: 5 μM for Bay, 10 μM/mL for p38, 25 μM/mL for JNK, and 10 μM/mL for MEK. Prior to PIC treatment, Bay was treated for of 3 h, while p38 and JNK inhibitors were applied for 1 h, and MEK inhibitor was treated for 18 h.
RNA from DF-1 cells was isolated using the Pure-link MiniRNA Extraction Kit (Invitrogen, Carlsbad, CA, USA). In preparation for real-time quantitative polymerase chain reaction (RT-qPCR), 1 μg of total RNA was utilized for cDNA synthesis, which was carried out with the Rever Tra Ace-α-first strand cDNA synthesis kit from Toyobo (Osaka, Japan).
For the analysis of chFABP4 expression, quantitative real-time-PCR (qRT-PCR) was performed with SYBR green supermix using CFX96™ IVD Real-time PCR System (Bio-rad, Hercules, CA, USA). The sequences of the chFABP4 primers were 5’-CTG GCC TGA CAA AAT GTG CG-3’ for the forward primer and 5’-GTG TGC CAC TCC TGT CTA GGG TT-3’ for the reverse primer. Chicken interleukin-8 like 2 (ChIL8L2) primers were 5’-CCA AGC ACA CCT CTC TTC CA-3’ (forward) and 5’-GCA AGG TAG GAC GCT GGT AA-3’ (reverse). The PCR conditions were as follows: an initial step at 94°C for 3 minutes; 39 cycles at 94°C for 10 seconds, 60°C for 30 seconds, and 72°C for 30 seconds; and a final step at 72°C for 10 minutes. Dissociation was performed at 0.5°C increments from 55°C to 95°C for over 25 minutes. Relative quantification analysis was performed using the comparative Ct (2(−ΔΔCT)) method (Livak and Schmittgen, 2001). The expression of glyceraldehyde-3-phosphate dehydrogenase (GAPDH) was used as endogenous control for the detection of mRNA expression levels. The sequences of GAPDH primers are: 5’-TGC TGC CCA GAA CAT CAT CC −3’ for forward primer and 5’-ACG GCA GGT CAG GTC AAC AA −3’ for reverse primer.
The results were presented as the mean±standard deviation of at least three independent experiments. Statistical significance was evaluated using Student’s t-test. Compared to the vehicle control, P<0.05 were considered significant. The difference among each value was determined by Tukey’s test with alpha<0.001.
RESULTS
In this study, our primary objective was to investigate the influence of TLR3 signal activation on the transcription of the chFABP4 gene in chicken DF-1 cells. To achieve this, we conducted two sets of experiments. First, we assessed the impact of various doses of PIC (0.1 μg/mL, 1 μg/mL, 5 μg/mL, and 10 μg/mL) on DF-1 cells over a 24 h period. We observed that IL8L2 expression increased in a dose-dependent manner up to 10 μg/mL (Fig. 1A) as in our previous study (Jang and Song, 2020). We observed a dose-dependent increase in chFABP4 mRNA expression up to 5 μg/mL, with a slight decrease at 10 μg/mL, as depicted in Fig. 1C (P< 0.001).
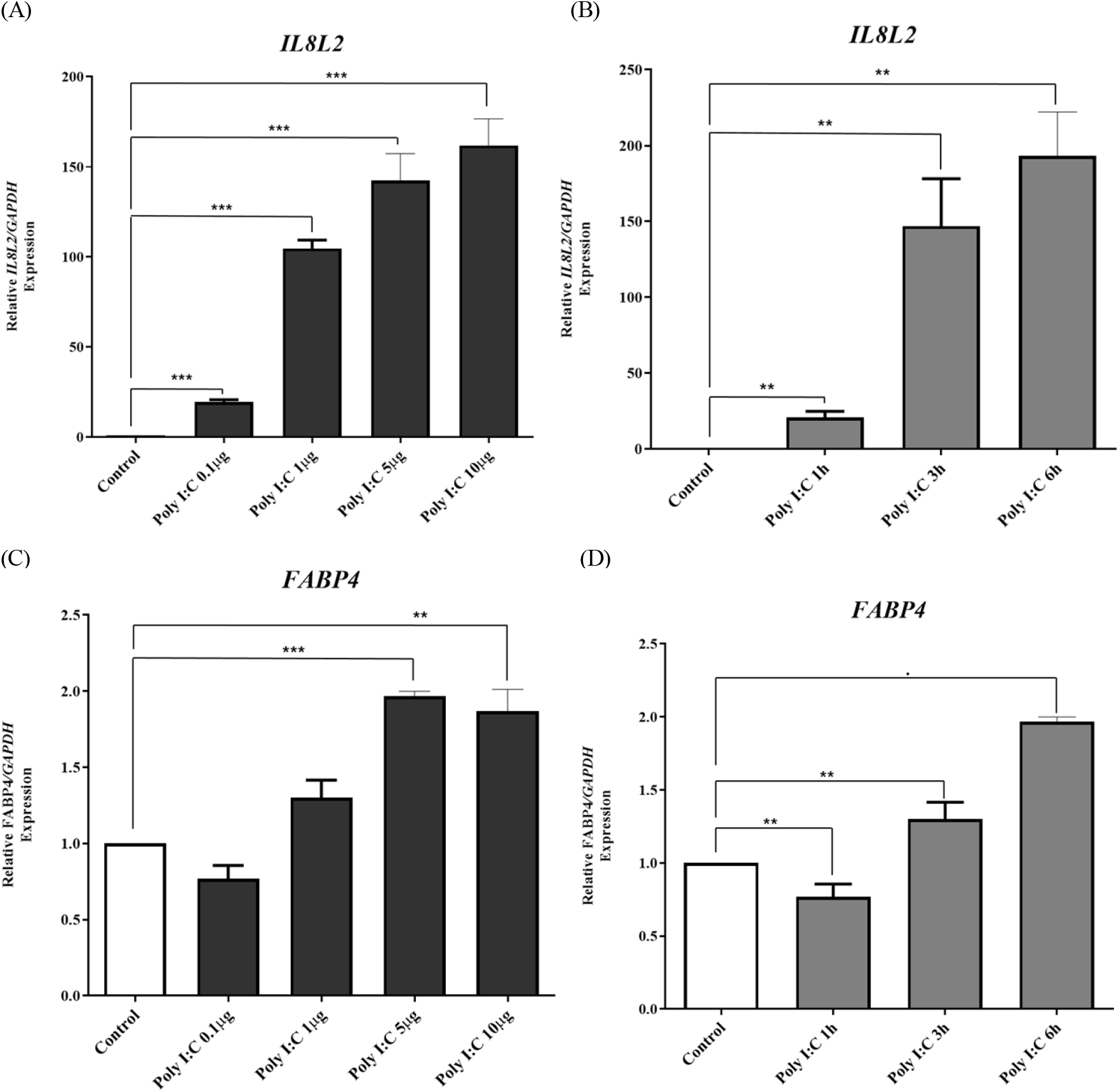
Subsequently, we treated DF-1 cells with 5 μg /mL of PIC for different durations, specifically 1 h, 3 h, and 6 h. Notably, both chIL8L2 and chFABP4 mRNA expressions increased in a time-dependent manner, as illustrated in Fig. 1B and Fig. 1D. These findings collectively indicate that chFABP4 mRNA levels are responsive to TLR3 activation via PIC, suggesting its potential involvement in the TLR3 signaling pathway or other associated cellular responses.
To test whether the NF-κβ transcription factor pathway is involved in chFABP4 transcription during TLR3 activation, we treated DF-1 cells with Bay 11-7085, an NF-κβ inhibitor, and analyzed mRNA expression of chFABP4 as well as chIL8L2 (Fig. 2B). As a result, inhibition of NF-κβ transcription factor pathway did not alter the transcription of chFABP4 whereas chIL8L2 transcription was suppressed significantly, suggesting NF-κβ transcription factor pathways are dispensable for chFABP4 transcription (Fig. 2B), whereas this pathway is indispensable for chIL8L2 transcription (Fig. 2A).
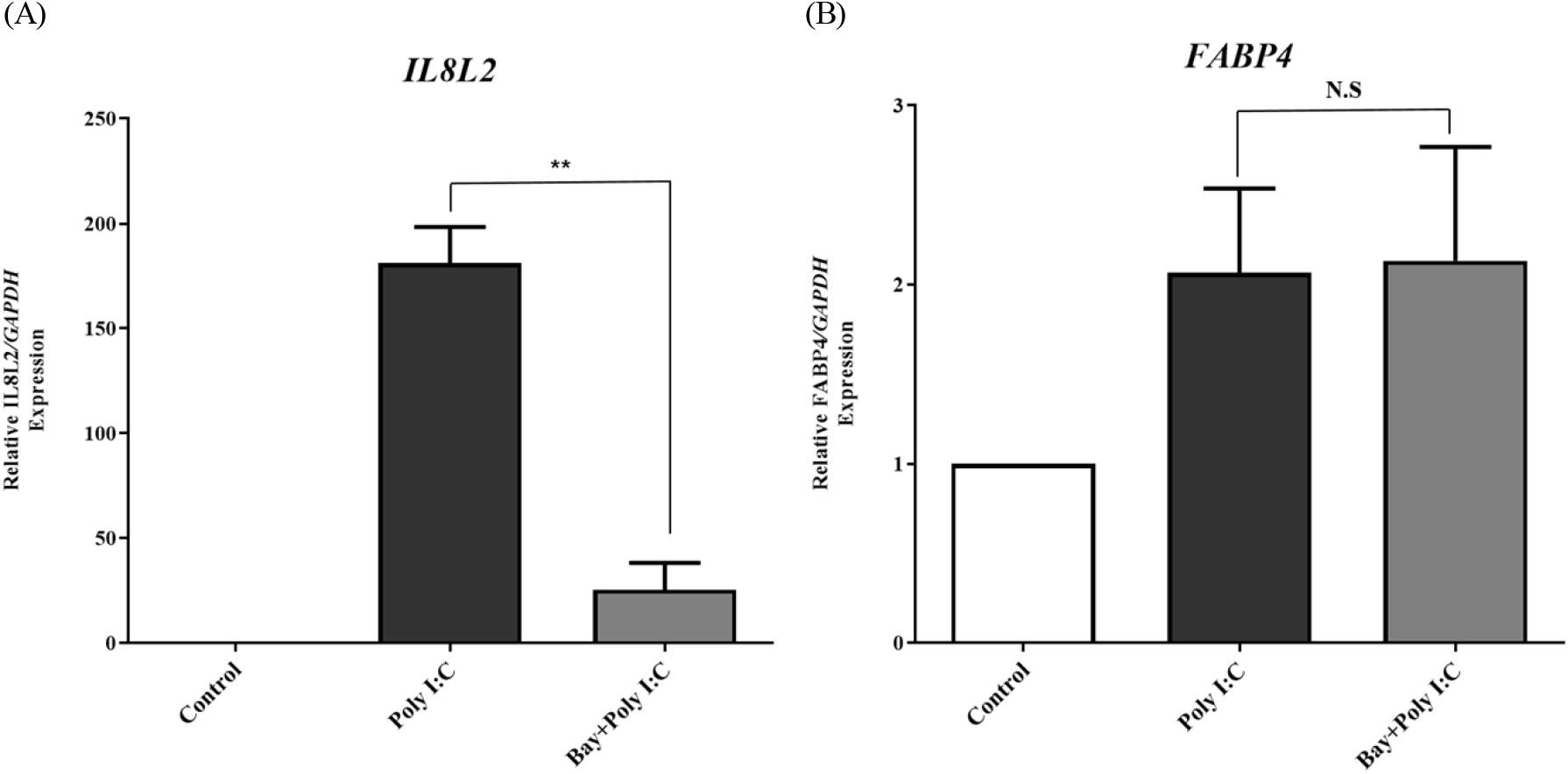
To explore the potential involvement of MAPK pathways in chFABP4 transcription during TLR3 activation, DF-1 cells were subjected to MAPK inhibitors, specifically SB203580 (a p38 inhibitor), SP600125 (a JNK inhibitor), and PD98059 (a MEK inhibitor), as previously described in our study (Park et al., 2023). Subsequently, we analyzed the mRNA expression of both chFABP4 and chIL8L2 as shown in Figs. 3A and 3B. In line with our previous study, the transcription of chIL8L2 exhibited significant downregulation when each of the MAPK signaling pathways was inhibited (Fig. 3A). Indeed, the regulation of chFABP4 transcription by MAPKs exhibited a unique pattern in the study. When JNK was inhibited in DF-1 cells, it resulted in a decrease in chFABP4 expression. In contrast, inhibition of p38 led to a significant upregulation of chFABP4 expression, indicating that p38 normally suppresses the transcription of chFABP4 during TLR3 activation. Notably, MEK inhibition did not have a substantial impact on the expression of chFABP4.
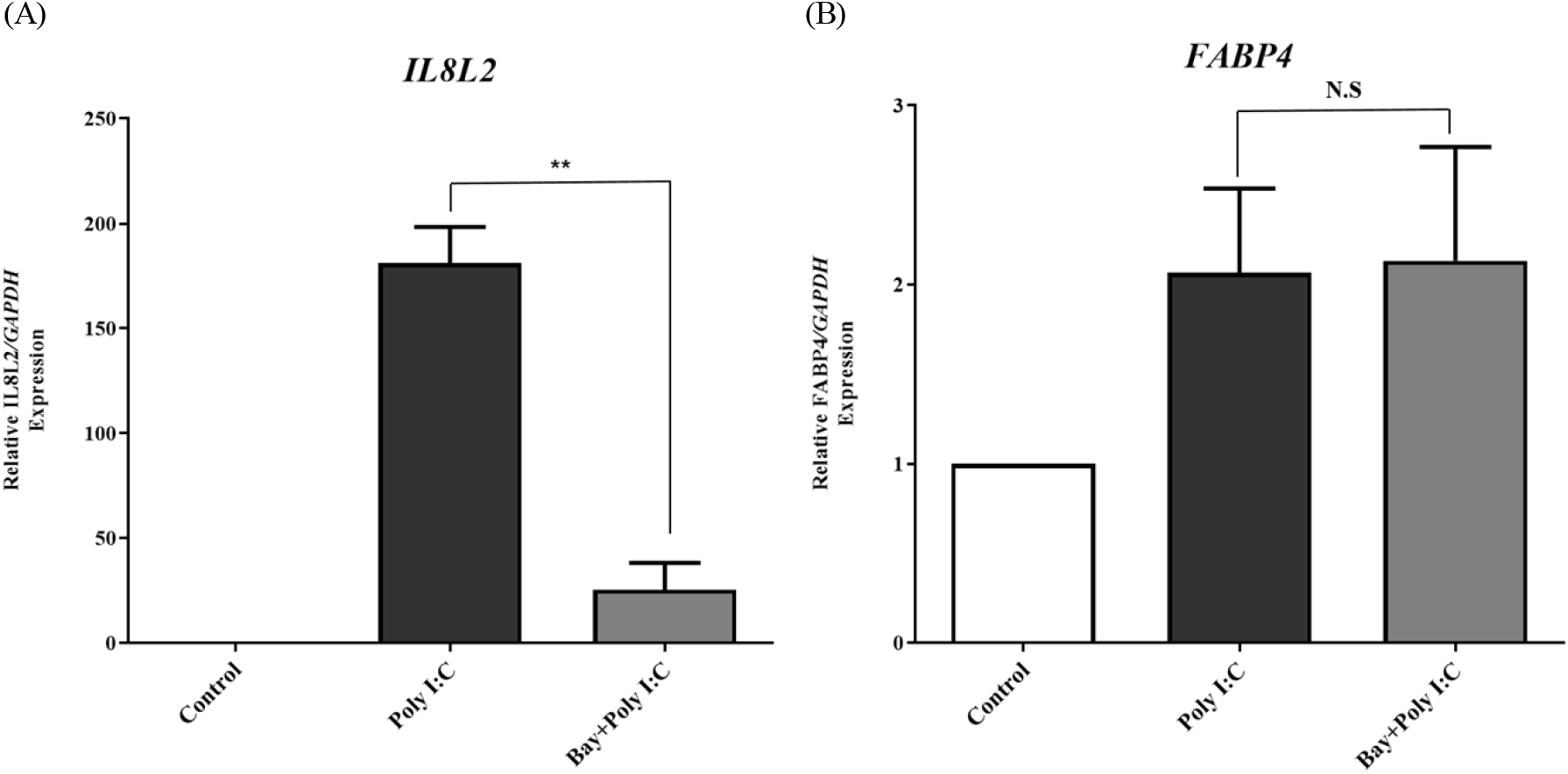
DISCUSSION
Previous studies have emphasized the critical role of FABPs in lipid transport and metabolic responses across various tissues, with distinct FABPs found in specific tissues and organs, such as liver FABP (L-FABP), intestinal FABP, heart FABP, adipocyte FABP, epidermal FABP, ileal FABP, brain FABP, and testicular FABP in mammals (Zimmerman and Veerkamp, 2002; Qigui et al., 2009). In avian species, the liver plays a significant role in de novo lipogenesis (Leveille et al., 1975; Shrago and Spennetta, 1976; Wang et al., 2017) and L-FABP has been identified as a genetic marker for fatness traits within the chicken QTL region (Wang et al., 2006). The role of FABP4 can extends beyond lipogenesis and adipogenesis regulation to include its involvement in immune responses following diseases or inflammation. Elevated circulating FABP4 levels have been associated with metabolic and cardiac dysfunction (Furuhashi et al., 2014). Furthermore, a positive link between FABP4 and plaque instability in carotid atherosclerosis, connected to the presence of CD68, CD163, and CD4, suggesting a vital role for FABP4 in the inflammatory lipid signaling process, potentially in conjunction with leukotrienes (Agardh et al., 2011). Additionally, gene expression profiles related to Wooden Breast disease (WBD) indicated an increase in FABP4 expression during vascular disease (Papah et al., 2018). Given the close association of FABP4 with the inflammatory response, there is a strong need for studies exploring the connection between FABP4 and signal transduction pathways involved in inflammatory responses in animals.
In our study, we investigated the transcriptional regulation of FABP4 in TLR3-stimulated DF-1 cells. We identified the chFABP4 gene through a transcriptome study in the kidneys of chickens exposed to varying calcium doses, as differentially expressed genes (DEGs) were identified in a previous study (Park et al., 2017). Activation of TLR3 in DF-1 cells with a synthetic ligand resulted in an up-regulation of FABP4 mRNA expression, indicating that the TLR3 signaling pathway regulates the transcription of chFABP4. Importantly, the activation of both TLR3 and TLR4 led to an up-regulation of FABP4 expression. However, it is particularly noteworthy that FABP4 expression significantly increased in response to LPS stimulation (Kazemi et al., 2005).
In the context of lipid metabolism, various signaling pathways, such as MAPK, TGF-beta, and Wnt signaling pathways, have been shown to support PPAR signaling, as reported by Ma et al. in 2021 (Ma et al., 2021). Therefore, we conducted an analysis of FABP4 regulation by inhibiting NF-κB, p38, JNK, and MEK signaling pathways. Our findings revealed distinct expression patterns for FABP4. Specifically, inhibiting JNK resulted in a significant decrease in FABP4 expression, while inhibiting the p38 signaling pathway led to an increase in FABP4 expression. Conversely, FABP4 expression seemed to be independent of NF-κB and MEK inhibition.
Concerning inflammatory responses, it was evident that FABP4 transcription was reliant on the TLR4-JNK signaling pathway activation, especially during LPS stimulation in mouse microglial cells. The absence of FABP4 in this context had a substantial impact on microglial energy metabolism, resulting in a reduction of the pro-inflammatory cascade and phosphorylation of JNK (Kagawa et al., 2023). In macrophage cells, a positive correlation was noted between FABP4 and JNK, associated with the activation of the inflammatory response (Hui et al., 2010). Notably, our present study yielded different results in the case of p38 inhibition, where inhibiting the p38 signaling pathway unexpectedly up-regulated FABP4 expression. Further research is needed to comprehensively understand the regulation of FABP4 expression by other signaling pathways, such as p38, and MEK.
In summary, these results underscore the intricate regulation of chFABP4 expression during TLR3 activation, which is influenced by the interplay of various MAPKs pathways. Specifically, JNK and p38 exhibited opposing effects on chFABP4 regulation, implying that the role of MAPKs in controlling chFABP4 is complex and involves multiple pathways. The specific pathway activated may vary based on the cellular context and signaling cues. Additional studies are warranted to thoroughly investigate these complex interactions. Further studies are required to fully understand these relationships.